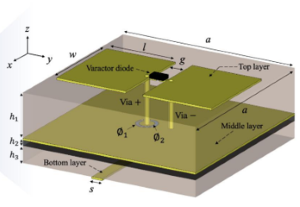
The ability for the metasurface to change its scattering property as a function of time opens new possibilities for the metasurface as the ultimate electromagnetic wavefront manipulation device. We perform fundamental and practical research in developing reconfigurable time-varying meta-atoms and demonstrating novel metasurface functionalities. Some example metasurfaces include adaptive wave redirection surfaces and time-modulated momentum manipulation surfaces. Exciting potential applications are envisioned reconfigurable intelligent surfaces for next-generation communication systems.
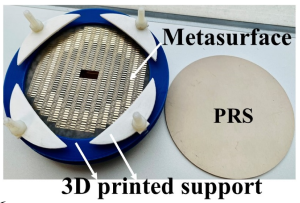
Most contemporary metasurfaces are either narrowband (they only work over a narrow bandwidth) or dispersive (their properties, such as scatter directions and focal lengths, change with frequency). We investigate “true-time-delay” metasurfaces (TTD-MS): metasurfaces which produce a constant delay in reflection and/or transmission, such that the radiation direction, reflection delay and/or focal length of metasurface can remain constant over a wide bandwidth. Applying discretized and Huygens element concepts to the TTD-MS, we arrive at interesting and attractive novel metasurface antennas and lenses.
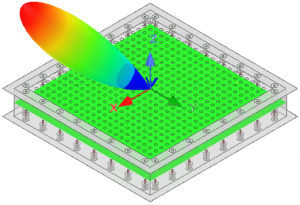
The metasurface, as a tool providing ubiquitous control in manipulating electromagnetic waves, modifies the radiation profile of an antenna with endless properties. Within this broad area, our group examines interesting combinations whereby the metasurface resides within the near-field of a source antenna and modifies its waveform, achieving desirable waveform properties unattainable by the antenna alone. Two examples include the flat wideband ORA where a metasurface replaces a curved ground plane, and a cylindrical metasurface which enables 360° azimuthal beam steering from a single planar phased array.
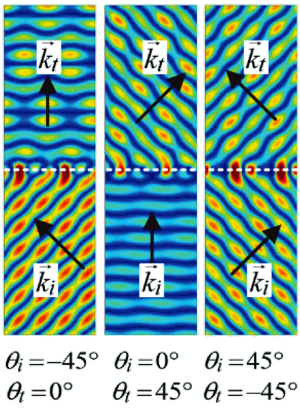
A Huygens’ metasurface is an artificially engineered surface which provides sufficient electric and magnetic response to turn an incident electromagnetic wave into a desired reflected and/or transmitted wave. Up till now, most metasurfaces are designed as a surface with continuous variations in their electromagnetic properties. However, in recent works, we have shown that coarsely discretized metasurface elements may actually make better metasurfaces than smoothly discretized ones. In some cases, using coarsely discretized elements can improve the metasurface performance and reduce its fabrication tolerance at the same time. An ongoing project will be charted to demonstrate the simple fabrication of this discretized Huygens’ metasurface at terahertz and optical frequencies: at these frequencies, it is highly non-trivial to fabricate and align traditional metasurface elements due to their small sizes. Successful demonstrations showing simplified fabrication and the versatile applicability of the discretized Huygens’ metasurface should pave way towards building practical high-frequency electromagnetic surfaces, which can be a very powerful electromagnetic wave processing tool, usable in beamforming, switching / routing, imaging and display engineering.
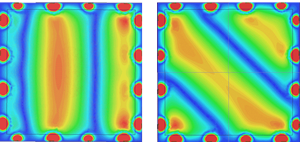
A lot of work has been performed on the design and implementation of planar metasurfaces. However, much greater possibilities await us when we wrap a metasurface around an area of interestc. Indeed, when one surrounds an area with an active metasurface that generates both electric and magnetic dipoles, one can generate an arbitrary waveform inside that area, as predicted from the fundamental electromagnetic equivalence principle. Very recently, we have performed experiment using what we call a Huygens’ box: a rectangular metallic cavity lined by a simple active Huygens’ metasurface. By properly exciting the Huygens’ metasurface, we demonstrated the generation of plane waves that travel in arbitrary directions within a rectangular metallic box, but disappear once they reach the edge of the box, with no reflections. Building on this, we intend on generating arbitrary waveforms inside an enclosed area by superimposing the aforementioned travelling waves. If successful, this technology may find impactful applications in imaging and therapy based on electromagnetic waves, a novel generation of antennas, and refined point-to-point indoor wireless propagation, just to name a few.
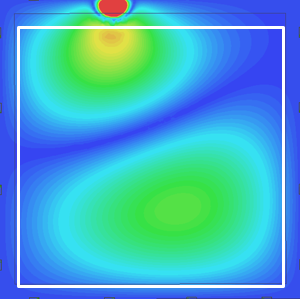
We investigate what happens when we excite arbitrarily designed electric and magnetic currents along the edge a metallic cavity using a closed active Huygens metasurface. We find that with appropriate excitations of such currents we can generate travelling plane waves within the cavity. These plane waves form “unconventional modes” which are disallowed by the physics of the cavity (in the absense of sources); moreover, using these unconventional modes one can synthesize arbitrary waveforms - including super-resolution waveforms - within the confines of the cavity. Forming a super-resolution focus within the cavity can be of dramatic benefit to applications like hyperthemia therapy (the selective ablation of malignant cells within the body) and wireless power transfer. Currently, we are progressing towards a first experimental demonstration of this phenomenon.
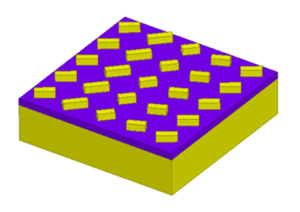
When light is reflected from a surface, in general it would encounter a loss in intensity and a change in phase. In this project we develop a surface which engineers light reflection at will - a surface for which the reflection loss and the reflection phase change can independently and arbitrarily tuned at sub-wavelength spatial resolution. With such tunability one can design at will the direction, strength and shape of a light beam which is reflected off the surface. We show (somewhat surprisingly) that such ultimate enginnering of light reflection can be acomplished by simply tuning the length and axis angle of a golden nanorod placed atop a metal-backed silica layer. Firstly, we show that under proper tuning this popularly studied nanostructure can operate as a Huygens source. From this perspective, we then show that by sweeping the aforementioned parameters one can obtain an arbitrary control of the reflection amplitude and phase with minimal compulsory loss. Currently we are working towards the construction of a Huygens metasurface which will serve as the super-resolution filter inside the next-generation Optical Super-Microscope (Please see below for a description on the Optical Super-Microscope project).
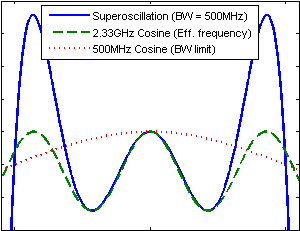
What is a Superoscillation?
Superoscillation refers to a phenomenon whereby a waveform oscillates faster than its highest constituent frequency component across a finite interval. With the local availability of wave components which oscillate faster than traditionally thought to be possible, one can form a sharper focus with electromagnetic waves, and construct images with higher resolutions. In my PhD project, I investigated ways to circumvent diffraction limitations with propagating waves using the phenomenon of superoscillation. I tried to understand superoscillation through its relationship with superdirectivity and found that the two phenomena are dual to each other. Leveraging this duality, I have adapted existing antenna design methods to design superoscillatory electromagnetic waveforms. These methods provide practical handles on waveform parameters such as the focal width, and sidelobe levels within and without the superoscillatory region.
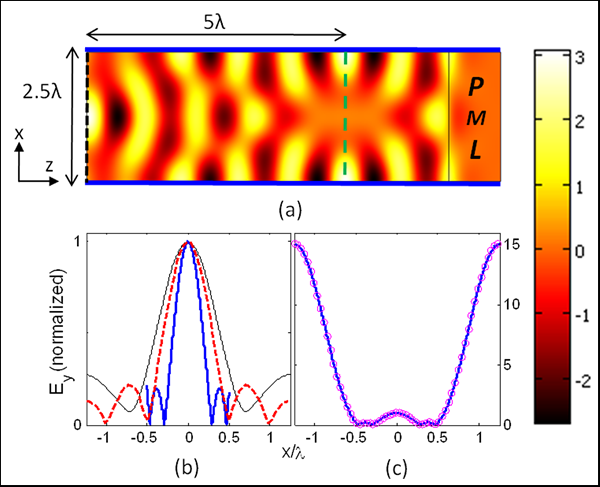
Sub-Diffraction Focusing at Microwave Frequency
Using my adapted design procedure I have demonstrated sub-diffraction focusing of microwaves at a five-wavelength working distance – 10-fold increased from the working distances of evanescent-field-based focusing systems. In my demonstration focusing occurred in a rectangular waveguide: five current sources excited a designed combination of three modes within a rectangular waveguide, which superimposed to form the superoscillation waveform after five wavelengths of propagation. Experiments at a frequency of 3GHz confirmed the presence of a sub-diffraction focus, whose width was reduced to 75% of that for a diffraction-limited waveform. This demonstration of sub-diffraction focusing away from the device’s evanescent near-field serves as a solid step towards performing sub-diffraction imaging.
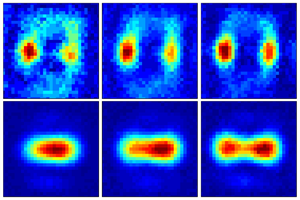
Optical Super-Microscope
Building upon my work in the microwave regime, I have demonstrated the Optical Super-Microscope (OSM), which is a 2D sub-diffraction imaging device at optical frequencies. The OSM achieves sub-diffraction imaging through a 4F imaging system, where a multiplicative filter at the Fourier plane implements a superoscillatory Green's function for the imaging system. As a result, the OSM achieves far-field, sub-diffraction optical imaging without the need for fine scanning, data post-processing or object pre-treatment. With a proof-of-principle prototype, I have reported a point spread function with a spot size clearly reduced from the diffraction limit, and demonstrated corresponding improvements in two-point resolution experiments. The OSM can be used in a wide variety of imaging applications beyond the diffraction limit, including real-time imaging of moving objects.
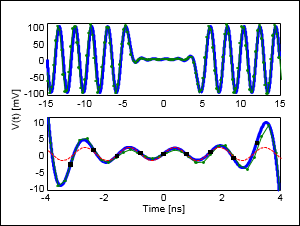
Time-Domain Superoscillations
I also apply my design procedure to construct temporal superoscillation waveforms – waveforms which contain temporal variations more rapid than their highest frequency components. Using an arbitrary waveform generator with 500MHz bandwidth, I have constructed two special superoscillatory waveforms: one which contained local oscillations at 650MHz, and another which contained a temporal peak compressed 47% beyond that of a 500MHz sinc pulse. To the best of my knowledge, these were the first temporal superoscillatory electromagnetic waveforms ever demonstrated. Currently I am working towards applying such superoscillatory waveforms to improve the range resolution of a radar system.
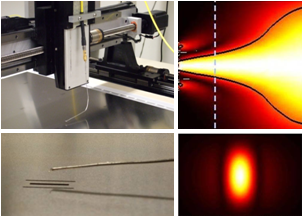
The holography-inspired metascreen is a metallic surface which provides a subwavelength electromagnetic focus at greater image distances than a point source probe. I began this project with an aim to record a hologram of the near-field of a point source, and to reconstruct it in a way that accounts for, and preserves, contributions from its evanescent components. As a result, I derived a spatially varying transmission screen (analogous to the hologram surface) which transforms an incident plane wave into a near-field point source. I then proposed a simple resonant slot antenna array, which effectively approximated the rapidly varying transmission amplitudes of the varying transmission screen, and thus achieved sub-wavelength focusing. A prototype metascreen was used to focus an incident83GHz wave to a spot width 75% of the diffraction limit, at a distance of 0.15 wavelengths away from the transmission screen. Such a screen provides a greater working distance compared to the conventional near-field probe. Moreover, resonant effects lead to extraordinary electromagnetic transmission across the slot apertures, and allow the formation of a focus of stronger intensity than the incident wave. I have also shown that this efficient resolution and power performance does not degrade with the introduction of metallic loss, thus distinguishing this screen from the loss-limited metamaterial superlens.
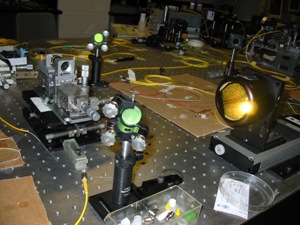
Optical signal processing and scientific instrumentation would benefit greatly from an efficient means of tuning the temporal profile of the optical signal. In my Bachelor thesis project, I have developed and implemented an adaptive algorithm to design fiber Bragg gratings, such that, when operated in reflection mode, they perform arbitrary shaping to an incident optical pulse. The algorithm designs gratings in the strong linearly chirped regime, allowing a relatively broad reflection band (about 30 nm on a 1550nm carrier), suitable for shaping optical pulses in the 10ps regime. Since the pulse shaper is performed in fiber, the resultant device is simple and power efficient compared to free-space pulse shaping solutions which existed prior to my work. I demonstrated this method by shaping a 1ps (3dB intensity) Gaussian pulse into 10ps and 20ps square pulses and triangular pulses, with power efficiencies of over 80%. To my knowledge this was the first passive pulse shaping method to achieve such high power efficiency levels.